Our planet holds a vast reservoir of energy that could sustain the current global population for 13 million years. We’re talking about nuclear fusion. Theoretical physicist Steve Cowley, one of the leading advocates of this innovation–along with many other scientists–sees this technology as the most promising alternative to both fossil fuel consumption and conventional nuclear fission.
In this post, we'll explore several important aspects of nuclear fusion, delving into its potential, its challenges, and its environmental implications.
Gyrokinetic Theory Allows Us to Be Optimistic
Currently, the challenges posed by nuclear fusion have more to do with engineering than with physics. Although the first forays into fusion began approximately five decades ago, those early Tokamak reactor designs stumbled upon an obstacle that was insurmountable with the resources available at the time: magnetic confinement.
Preserving a magnetic field capable of controlling the turbulences of plasma–which, in fusion, is a gas that contains deuterium and tritium nuclei at temperatures of up to two hundred million degrees Celsius–is no easy task. To solve this challenge, it was necessary to develop models capable of predicting with absolute precision the behavior of the plasma, so that it would be possible to design a very stable magnetic field that was capable of preventing this gas from touching the walls of the container isolating it from the outside.
The problem is that when physicists began to work on nuclear fusion, they lacked the necessary tools to create models capable of predicting how plasma behaves inside the reactor. The early experiments were frustrating. Their results were radically different from what the models of the time predicted, and stable, enduring fusion still seemed far away, despite the steady advances in reactor design.
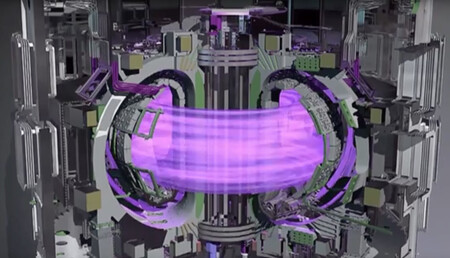
Fortunately, the study of the interactions of charged particles and plasma began to change drastically two decades ago thanks to the advent of modern supercomputers, which offer the necessary computing power to tackle problems of this kind. With them, it was possible to develop gyrokinetic theory, which can be defined as the set of mathematical models that describe the behavior of plasma at the temperatures found inside nuclear fusion reactors.
For the last 20 years, the computing power of supercomputers has continued to increase. And, moreover, physicists have developed more sophisticated mathematical models, so today it's possible to describe with great precision how plasma will behave during fusion. If you think about it, it's exciting how, despite lacking this knowledge, the technicians at JET (Joint European Torus) in the United Kingdom managed to sustain the nuclear fusion reaction for two seconds in 1997, obtaining 16 megawatts of fusion power–or, put in a slightly more manageable unit, 16 million watts.
With this precedent, and considering the huge impact that the development of supercomputers has had on gyrokinetic theory, there are great expectations surrounding what the scientists and engineers involved in the ITER reactor will achieve once it’s up and running. The governing council of this project has set 2025 as the year in which the first plasma tests will be carried out in the reactor, so the advances introduced by gyrokinetic theory in recent years–as well as those that come before that time–should make a difference.
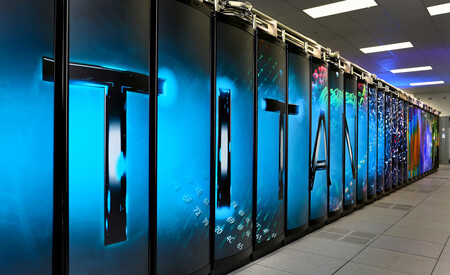
However, executing these mathematical models on supercomputers has two other advantages that must not be overlooked: cost reduction and time savings. Building a nuclear fusion reactor, as anyone can guess, isn't cheap. According to the global consortium responsible for its design and construction, ITER will cost more than $20 billion, and that’s not counting maintenance costs, which will also be considerable.
Here, supercomputers and gyrokinetic theory come into play again. Thanks to these two resources, scientists can modify the parameters of the mathematical model to know what effect that variation will have on the behavior of the plasma; in this way, they can figure out what modifications must be introduced in the reactor, with a very precise notion of the effect they will have on the fusion reaction.
Of course, these calculations are very complex, and there is a cost in terms of the energy consumption of the supercomputers. Still, it’s clear that this expenditure is much lower than what would be required if they were to implement these modifications in the reactor without having a reasonably accurate idea of what to expect from them.
Muon Fusion: The Perfect Nuclear Fusion, Although Far from Profitable
Some of the most notable scientific advances are the result of chance–although, of course, this is a field in which chance appears when you open the door to it. This is worth mentioning because, curiously, the method that scientists consider most efficient for carrying out nuclear fusion from the perspective of the temperature was predicted by theoretical physicists Andrei Sakharov and Frederick Charles Frank–the former Russian, the latter British–in the 1940s. A confirmation of their finding would come a little later, in 1956, through an experiment carried out by Luis Walter Álvarez, an American experimental physicist of Spanish descent, at Berkeley.
A brief explanation before continuing: A bubble chamber is a container, usually filled with liquid hydrogen slightly below its boiling point, designed to study particles with an electric charge. Its operation is relatively simple: by introducing a charged particle, the liquid reaches its boiling point and the particle leaves a perceivable trail of bubbles that serves to study some of its properties, like its mass or its charge.
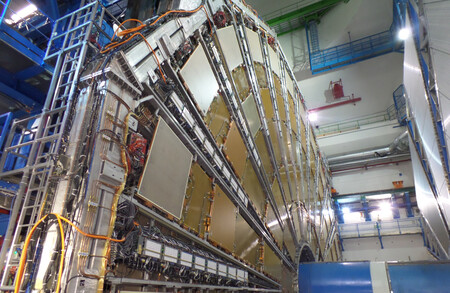
Now, L.W. Álvarez was using the university’s bubble chamber to study the interaction of muons with the liquid hydrogen contained in it and, unintentionally, stumbled upon muon-induced fusion. A muon is an elementary particle that has the same negative charge as an electron, but differs from it in two very important properties: its mass is two hundred times greater than that of the electron, and it revolves in an orbit that is two hundred times closer to the atomic nucleus.
If we put a muon in a container with a nucleus of deuterium and another of tritium, the muon will take the place of an electron, but it will be much closer to the proton of the nucleus (deuterium and tritium are isotopes of hydrogen and have a single proton in the nucleus) than the original electron was.
Since the muon has a negative charge and the proton’s charge is positive, given its much greater proximity to the electron's orbit, the charge of the proton is neutralized, so another nearby proton may approach the proton surrounded by the muon. If the charge of the latter has been neutralized and both protons come close enough, a strong nuclear interaction could come into action, causing the fusion of both nuclei, with the subsequent release of energy.
All this simply means that if we place a mixture of deuterium and tritium in a container and introduce a muon, it will cause the fusion of a deuterium nucleus and another of tritium. A lot of energy will be released, and the muon won’t be consumed. In fact, one of these particles can be involved in up to two hundred fusions before it disintegrates. And the advantages of muon-catalyzed fusion do not end here.
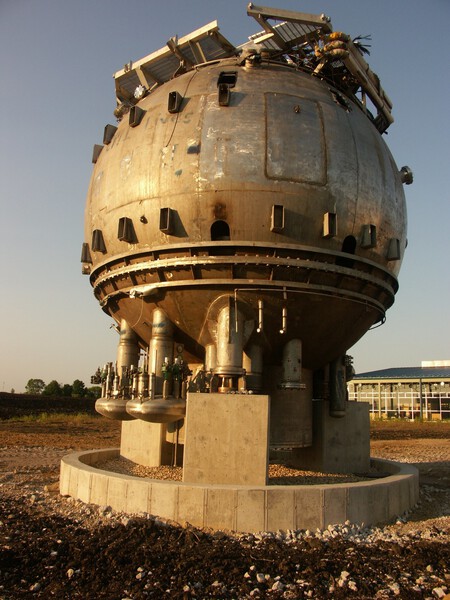
To fuse deuterium and tritium using a muon as a catalyst, the nuclei of the first two elements don’t need to be at the 200 million degrees Celsius required for nuclear fusion by magnetic confinement. It is enough for them to be at a temperature of approximately 500 degrees Celsius–which is nothing compared to those 200 million degrees. In fact, this is why this form of fusion is usually known as “cold fusion,” even though it is not really cold at all.
Now, everything’s peachy up to this point. However, two important restrictions prevent this fusion process from being profitable. For now, at least. First, muons disintegrate after reaching close to two hundred fusions. Also, in order to obtain a muon, we need to collide particles and invest approximately 200 times the energy we will obtain as a result of nuclear fusion in this process. Under these conditions, the process is clearly not profitable from an energy point of view.
The only way to achieve a positive energy balance during this process requires finding a way for muons not to disintegrate after those approximately 200 fusions, and for now, this possibility goes against fundamental physics. Even so, if someone were to find a way for a muon to last more than 200 fusions, the fusion induced by these particles would be profitable, and our energy needs would finally be met for the 13 million years that I mentioned at the beginning of this article. Unfortunately, this still belongs in the realm of science fiction.
The Environmental Impact of Nuclear Power
Everything seems to indicate that fossil fuels are not going anywhere–at least not for a while. Although we've been reading and hearing for a long time now that oil reserves will only last for a few decades, the experts are divided on the volume of the reserves of the countries that dominate this market, largely because many nations aren’t interested in disclosing their stock.
In addition, the emergence of fracking–a highly controversial technique due to its possible involvement in seismic movements, as well as its polluting potential–seems to be helping the U.S. to substantially increase its crude oil reserves. In fact, a report by the Norwegian consultancy Rystad Energy reveals that most of the oil reservoirs in America have not yet been found, so there could be much more oil than experts suspected a few years ago.
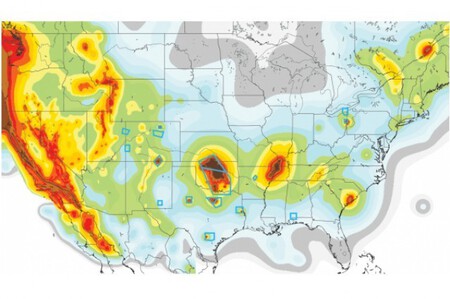
But that’s not all. Oil isn't the only fossil fuel that we use. We also get energy from natural gas, unconventional oils, shale gases, bituminous sands, and many other resources which seem to indicate that we don’t have to worry about hydrocarbon reserves, at least not in the medium term. In fact, Steve Cowley, the physicist who champions nuclear fusion, claims that fossil fuels can provide us with energy for at least two more centuries. He doesn't say that optimistically, though. He says it with dismay.
What might sound like good news at first, may not be so if we consider the impact that the burning of hydrocarbons at the current rate–or faster–will have on the environment for decades. Leaving aside the discussion about the influence of humanity on climate change, what is evident is that continuing to resort to fossil fuels for many more decades can be risky, even assuming that we still don’t have the necessary knowledge to quantify this risk and its possible consequences accurately.
It is precisely in this scenario that nuclear fusion gains relevance as the source of clean, safe energy that scientists hope it will be. Clean, because the product of fusion is a helium atom–which is stable, and therefore not radioactive–and a high-energy neutron. The latter will escape the magnetic field that confines the plasma and will end up colliding with the walls of the container, which will be coated with lithium with the aim of generating new tritium that will be reused in the fusion reaction.
This strategy poses a problem regarding waste: Those high-energy neutrons can collide with the nuclei of the container walls, degrading the material and making it radioactive. This is precisely what scientists in the IFMIF-DONES project are trying to solve, or at least mitigate. But no matter how bad it gets, waste from nuclear fusion represents a much smaller problem than that resulting from nuclear fission.
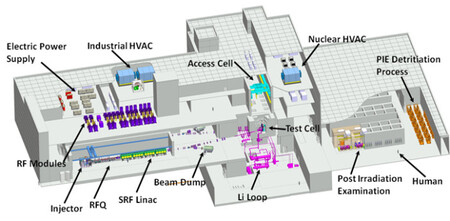
The radioactivity of the materials used on the container walls will be a thousand times lower than that of the plutonium obtained in current nuclear fission plants. In addition, its half-life is approximately 10 years, and in a century these materials would be completely harmless. As you can see, this scenario is much easier to defend than that posed by fission. Even so, technicians hope that the IFMIF-DONES project allows us to further reduce fusion waste.
On the other hand, to understand why nuclear fusion is safe, we only need to consider that, at any given moment, there is less than a gram of fuel inside the reactor. Even if the container has a volume of more than a thousand cubic meters, as is the case of the ITER fusion reactor. In case of an accident, all they would need to do is stop supplying fuel to the reactor, and the fusion would stop immediately.
In addition, the conditions necessary to carry out the reaction are so demanding in terms of temperature that any instability would halt the process–which, in addition, can't trigger any kind of chain reaction.
Energy and Human Development
Before concluding the article, it seems important to devote a few lines to the essential role that energy plays in human development. Steve Cowley began the conference where I had the opportunity to meet him by addressing this topic, before saying a single thing about physics and engineering. Because the effort that human beings are making to try to make nuclear fusion work makes sense if we really manage to use it to meet our energy needs. Not just those of the more developed countries, but also those of the poorest nations that are currently devastated by the lack of opportunities caused, among other factors, by the lack of energy.
To improve their standard of living, these people need to consume more energy than they currently have. That, anyone can understand. But, in addition, there are countless reports conducted by academic authorities and researchers that accurately relate the dependency between quality of life and the energy return on investment (EROI).
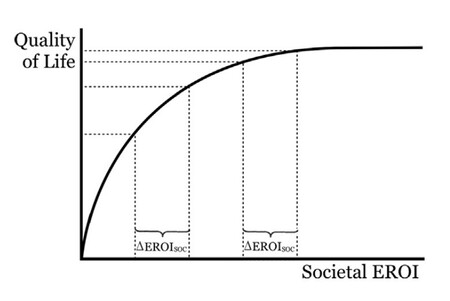
This rate relates the total energy we obtain from a source to the energy we must invest in the process to use that resource. The EROI is the quotient of these two values, so the ideal result would be greater than one, considering that we place the energy we obtain in the numerator, and the energy we invest in the denominator. A result greater than one reveals a positive energy balance, something that we were already able to achieve with nuclear fusion during the experiment carried out in JET in 1997.
It is possible that, when nuclear fusion becomes commercially viable, only a handful of countries will have the necessary technology to design and build fusion reactors. However, the fuel will be available to a very large number of nations as it will be extracted mainly from seawater, which will contribute decisively to democratizing nuclear fusion, and therefore, to making energy available to many more millions of people than today.
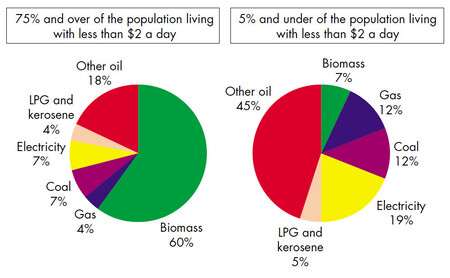
Deuterium is an isotope of hydrogen that is very abundant. In fact, we can find 34 grams in every cubic meter of seawater. The oceans are also a very important source of lithium, and, having these two elements, fusion is already possible; even if tritium is scarce and unstable, we only need very little of it, as we can obtain it within the fusion reaction itself.
Nuclear fusion is, without a doubt, one of the greatest scientific and technical challenges that humanity faces. But it is also one of the greatest opportunities within our reach; an opportunity to obtain the energy we will need in the future, to do it while respecting the environment much more than we currently do, and to make energy available to many more people, equalizing opportunities. If only many other technical innovations could allow us to have a dream like this.
Images | NASA | TDC | ORNL | IFMIF-DONES | Svdmolen
View 0 comments